Molecular Orbitals in Organic Chemistry
- Benjamin Hui
- Jan 25, 2019
- 5 min read
Updated: Feb 13, 2019
Atomic orbital theory states that electrons orbiting a nucleus have fixed energy levels, "quanta", and inhabit defined volumes of space around the nucleus called orbitals whose sizes and shapes are defined by the energy level of the electron. Under this theory, it is impossible to predict where exactly an electron is at any given moment of time. Simply put, an atomic orbital is a volume of space around the nucleus where there is a probability of finding an electron.
When atoms form molecules, atomic orbitals overlap to form molecular orbitals (MOs), which is the basis of a bond. Orbital conservation applies, whereby the number of MOs formed equals the number of atomic orbitals that overlap. For example, two orbitals overlapping forms two MOs - a bonding and an antibonding orbital. Both electrons are contained in the bonding orbital. The antibonding orbital is higher in energy and is empty. Molecules are made up of a complex interweaving of MOs, all with different energy levels, and because of this, electron densities vary through the molecular landscape. Some regions might be electron rich, and some poor.
MO theory was really confusing for me when I was first introduced to it, and frankly, it still is. Even simple molecules like oxygen and nitrogen contain rather complicated hierarchies of MOs, bonding and antibonding. When I recall the other molecules we looked at, like the cyanide anion, all I can say is that I'm glad I pursued organic chemistry. Compared to other areas of chemistry, MO theory in organic chemistry is more streamlined and has less considerations. Full MO diagrams are rarely employed when considering organic chemistry problems because organic molecules are a lot more complex. Only MOs involved in bonds that are broken or formed are looked at. Here's some of the more important stuff that we need to know.
1. Know the shape of the MOs
We know there are only two types of MOs: sigma and pi, and their antibonding counterparts sigma* and pi*. We also know how they form: Most sigma bonds are formed from the end-on overlap of various combinations of three kinds of hybridized atomic orbitals (sp^3, sp^2 and sp). This article assumes knowledge of how these orbitals arise. Pi MOs are formed from the side-on overlap of p-orbitals only. Hybridized orbitals do not participate in pi-bonding.
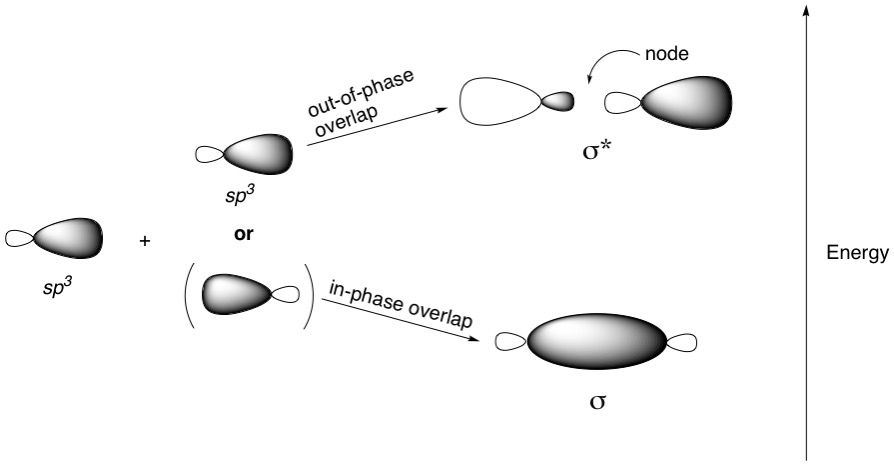
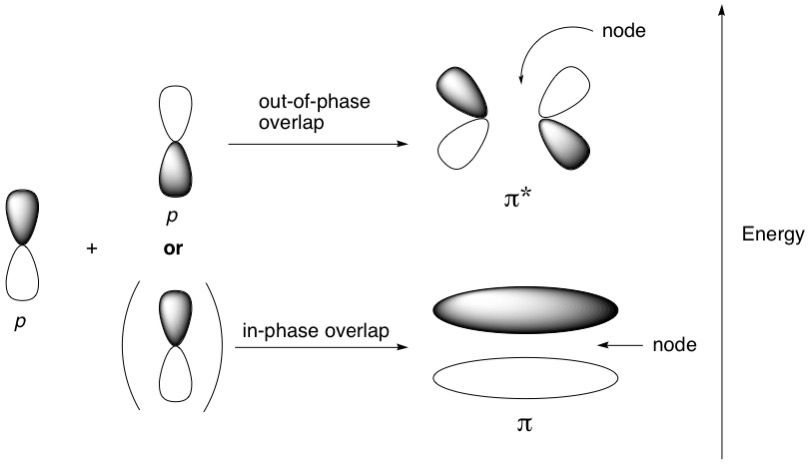
Recall that to break a bond, two electrons need to go into the antibonding orbital corresponding to that bond. For a single bond, this will reduce the bond order from 1 to zero, effectively cleaving that bond. Knowing what these MOs look like is important when considering reactions like the Sn2 substitution. For example, in the reaction of bromomethane with cyanide as the nucleophile, the shape of the sigma* demands that there can only be one vector of attack - straight on at the sigma* orbital lobe sticking out of the carbon atom. No other approach will do because the geometry would be incorrect and effective orbital overlap will not happen. This is called a back-side attack because the nucleophile approaches from behind the leaving group (Br) and boots it out of the door.
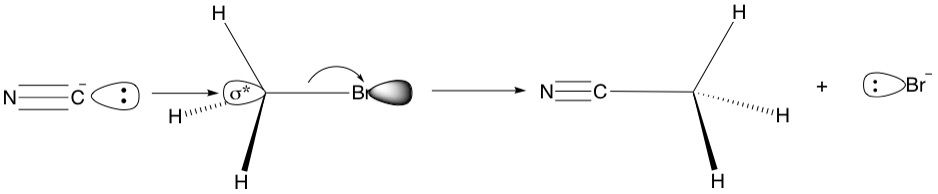
For a nucleophilic attack on an sp^2-hybridized carbon centre, such as a carbonyl group, the pi bond breaks. This means that the nucleophile must insert its lone pair into the pi* antibonding orbital, and just like breaking a sigma bond, the nucleophile needs to approach at a particular angle. Due to what a pi* antibonding orbital looks like, with its lobes fanning outwards, this angle is about 107 degrees and is known as the Bürgi-Dunitz angle. Note that this angle is pretty close to the ideal tetrahedral angle of 109.5 degrees. This makes sense because after the nucleophile attacks the pi* orbital, breaks the pi bind and forms a new bond, the resulting intermediate is tetrahedral in shape.
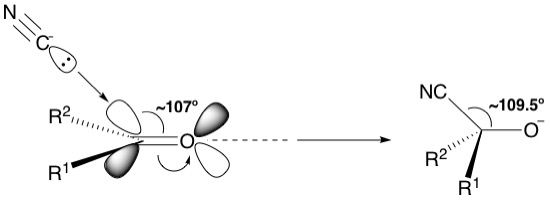
2. Conjugated systems and the HOMO-LUMO gap
At this point, we should already have come across conjugated systems. These are basically double bonds in series, separated by single bonds. These systems are special because all the p-orbitals making up the double bonds can now interact with one another. As a result, the electrons in these p-orbitals can now freely travel throughout this highway of interconnected p-orbitals. We say these electrons are delocalized. They are no longer confined to their parent atoms.
Another consequence of all the p-orbitals interacting is that there will now be more MOs formed. Consider butadiene, which has two conjugated double bonds. This means that four p-orbitals are interacting to give four MOs: two bonding and two antibonding. Also, four p-orbitals means four electrons, so these go into the two bonding orbitals. The four different MOs arise from the four different configurations that the four p-orbitals can come together, and contain an increasing number of nodes (regions with zero electron density resulting from out-of-phase overlap) from 0 to 3. The more nodes, the higher the energy of the MO. The HOMO (Highest Occupied Molecular Orbital) and LUMO (Lowest Unoccupied Molecular Orbital) as well as the energy difference between them (HOMO-LUMO gap) are all indicated on the diagram:
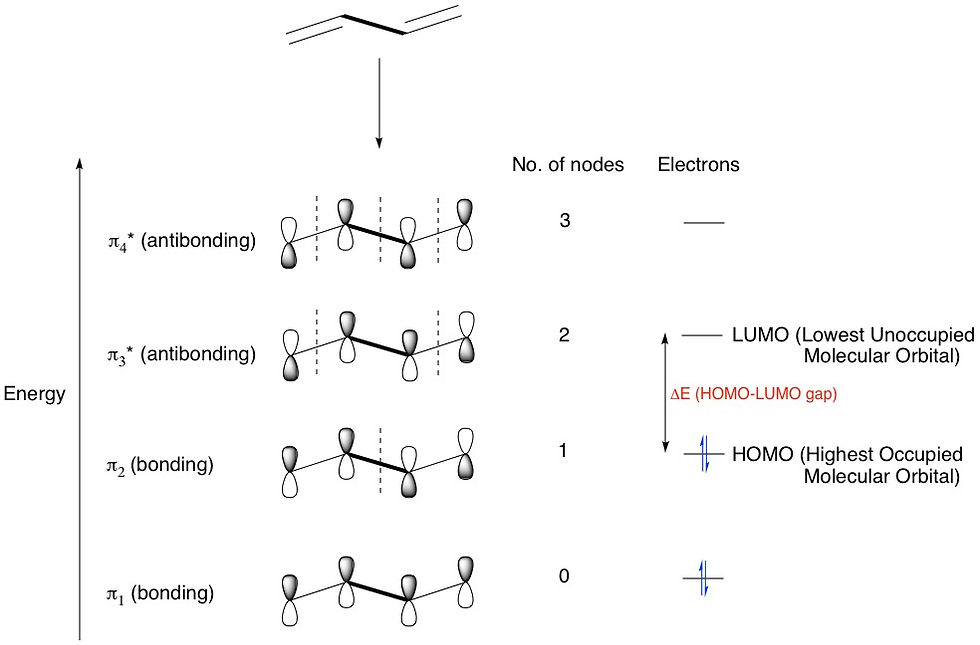
Let's add one more double bond and make it hexatriene. Now we have six p-orbitals overlapping in six different ways to form 6 MOs - 3 bonding and 3 antibonding. We're now beginning to notice a pattern: that the number of p-orbitals = the number of configurations = the number of MOs formed.
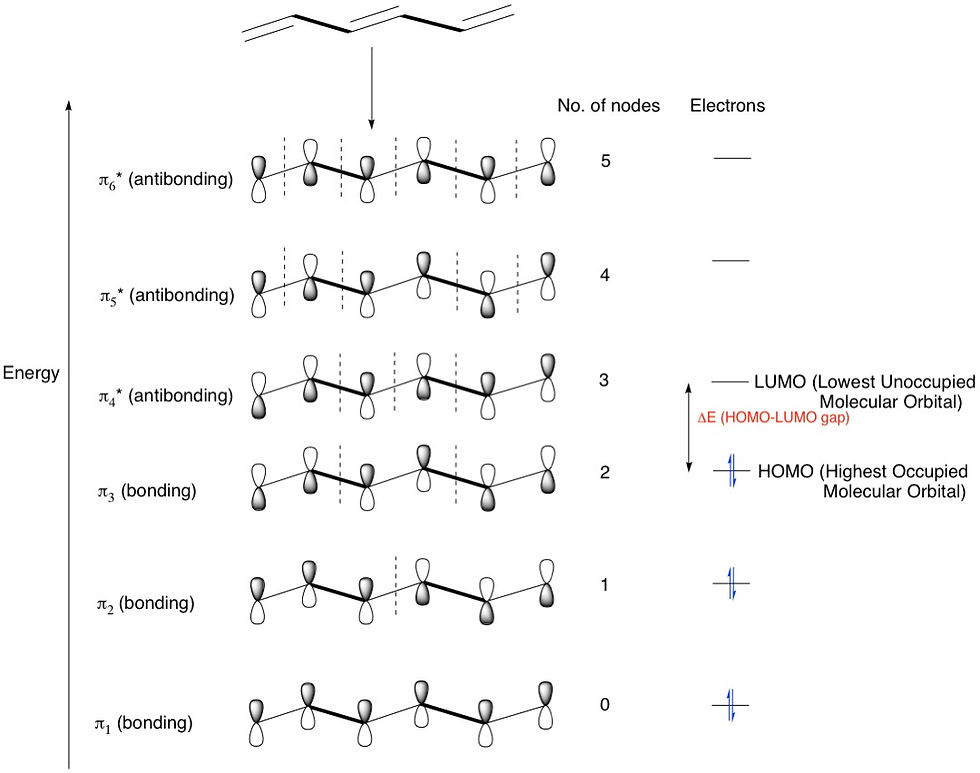
Another observation is that the HOMO-LUMO gap has decreased. This makes sense because the MOs, especially the higher energy antibonding ones, are now spread across 6 atoms instead of 4 for butadiene and therefore are more stable and hence have lower energies, bringing the HOMO and LUMO closer together. The trend therefore is that the more conjugated a molecule is, the smaller the HOMO-LUMO gap. Eventually, the gap starts corresponding to visible light wavelengths and the compounds start getting colours. Lycopene, for example, is the compound that gives tomatoes their red colour. This compound is highly conjugated, containing 11 double bonds!
So why is knowing about conjugation and HOMOs and LUMOs and gaps important? This is so that chemists can predict the reactivity of compounds by comparing their MO energy levels. With this information, we can predict whether or not a certain compound will respond to a certain reaction condition, or a reagent. Lycopene, for example, being highly conjugated, has a much lower HOMO energy than say, butadiene and therefore very unreactive with other molecules such as electrophiles. It really doesn't want to break that beautiful conjugation. The low LUMO energy as well makes lycopene unwilling to accept nucleophiles with their high energy lone pairs.
It is hoped that this article will allow some change of minds about the perceived difficulty of MOs in organic chemistry. With a little more reading up, MOs can be a joy to behold!
Коментарі